Welcome to the second issue of Volume 4 of PLANE WAVE, Verasonics’ Newsletter through which we share information about new products and technologies, emerging applications, conferences, training opportunities, and collaborations with researchers in ultrasound and ultrasonic technologies. We hope you find these newsletters informative and interesting, and welcome your suggestions for future topics.
New Products and Applications Technology Information Research Conferences and Training
Vantage Research Ultrasound Platform –
Enabling Innovation using Ultrafast Imaging
Background
Ultrasound has been used for medical imaging since the 1970’s. The conventional method to acquire images is to use focused beams to scan the region of interest by electronically shifting and phasing the transducer elements that are used for transmit and receive. While this method provides excellent image contrast, the fact that hundreds of individually-produced lines are required to reconstruct one single image limits the frame rate to less than 100 frames per second (fps).
In 1977, Bruneel et al. described the first application of “ultrafast” imaging. Their study allowed them to generate images at rates of up to 1000 fps. However, due to technological barriers at that time, implementation of their technique in an ultrasound scanner was not possible (Bruneel et al., 1977).
In the past two decades advances in electronics have made it possible to receive and store data from hundreds, even thousands of ultrasound elements simultaneously using software-based solutions rather than preconfigured hardware. This capability has given birth to what is now known as plane-wave imaging, which is the foundation for ultrafast ultrasound imaging as it is known today. The purpose of plane-wave imaging is to sonify the entire field of view using all elements in an ultrasound array at once, and then receive the backscattered signal returning from the medium, using all elements, and save it into memory. The ultrasound image is then beamformed using the contributions from each element in the array given the transmit and the receive paths, to produce pixel-oriented reconstruction (Daigle, 2012). In this manner, an image can be generated by one transmit-receive event, which allows for frame rates up to about 20 kHz for soft tissues.
The primary disadvantage of plane-wave imaging is loss in resolution and contrast in the acquired images. However, Lu et al. (2006) proposed adding different steered plane waves (or limited diffraction beams), coherently to improve resolution or incoherently to reduce speckle. Later, in 2009, Montaldo et al. demonstrated that 71 compounded plane waves (with an angle separation of 0.47° between waves) provided better SNR and contrast compared to multifocus line imaging, while improving the frame rate by a factor of 10.
Enabling Innovation using Ultrafast Ultrasound Imaging with Vantage
Verasonics Vantage™ Research Ultrasound systems enable users to transmit and receive plane waves at different angles at frame rates which are only limited by the speed of sound in the medium. Moreover, Vantage software provides beamforming and compounding capabilities that are required to reconstruct the ultrasound images. Figure 1 shows acquisitions performed using a Vantage 256 (only using 128 channels) with a 7.25 MHz, 128 element linear array transducer on a CIRS 040 phantom with different numbers of transmit angles. The image in panel A was acquired using only one plane wave steered at 0º, that is, parallel to the array. Panel B shows the image reconstructed using compounding of seven waves with the angular range for all multiple plane wave images -15º to 15º. The image in panel C was acquired using 41 plane waves steered from -20° to 20° in 1° increments. Lastly, panel D shows a conventional ultrasound image using 128 focused beams for image reconstruction. The corresponding frame rates for these imaging sequences (i.e., Panel A-D) are 12.5 kHz, 1.8 kHz, 0.3 kHz and 0.1 kHz, respectively.
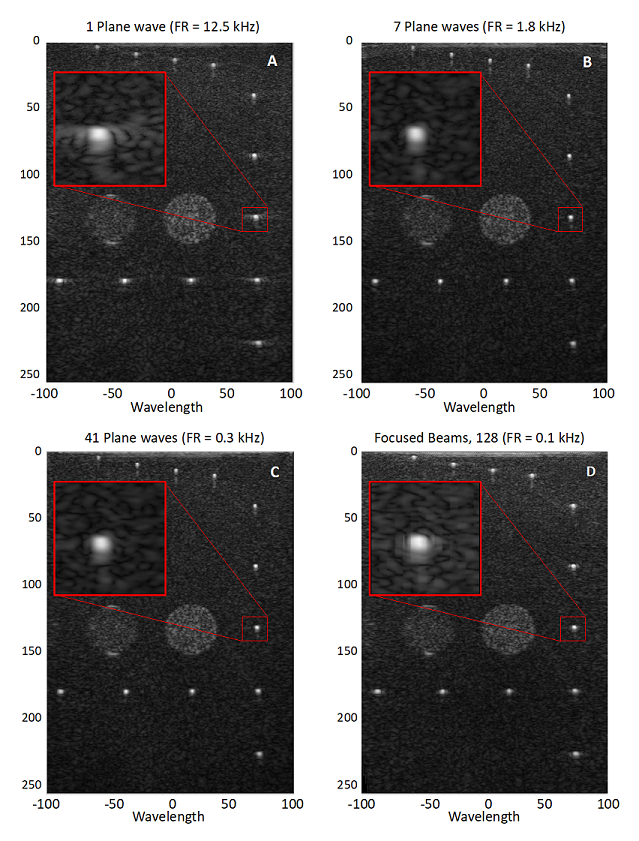
Figure 1: Comparison of the image quality for ultrafast imaging and focused beams. Panels A to C show ultrafast imaging with 1, 7 and 41 compounded angles, respectively. Panel D shows an acquisition with 128 focused beams. The differences in frame rate (FR) are shown at the top of each figure.
The development of ultrafast ultrasound imaging has opened the door to groundbreaking ultrasound modalities such as shear-wave imaging, functional ultrasound and ultrafast volume imaging, to name a few. In 1999 Sandrin et al. described the first work in transient shear-wave elastography, in which they mechanically created a shear wave that propagated in the tissue, and then used ultrafast ultrasound imaging to track the propagation of the wave. The ability to determine the speed of propagation of shear waves in biological tissue provided insight on the mechanical properties of the tissue. Later, in 2004, Bercoff et al. replaced the external mechanical stimulus with ultrasound radiation force to generate the shear waves and use the same transducer for ultrafast imaging (Bercoff et al., 2004).
Figure 2 shows the generation of a shear wave using ultrasound radiation force, and the subsequent visualization of the wave as it propagates through the medium using ultrafast imaging. These images were acquired with an L7-4 probe (with a center frequency of 5.2 MHz) connected to a Vantage 256 system (only using 128 channels) scanning a CIRS elastography phantom. The acoustic emission used to create the shear-wave source (i.e., push) consisted of a toneburst of 200 μs, focused at 50 wavelengths at 60 V. The transmit was done using the 37 center elements of the probe out of 128 elements. The visualization of the resulting shear wave was done by a single plane wave using 0º steering. In this case 50 images were acquired for a total acquisition time of 5.0 ms at a frame rate of 10 kHz. Even though the Vantage system is capable of acquiring data at up to 100 kHz per transmit-receive event, given the stiffness of the CIRS phantom, 10 kHz is enough to track the wave.
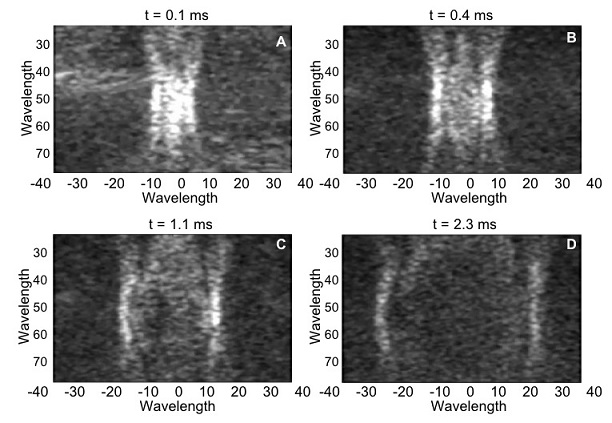
Figure 2: Shear wave propagation sequence on a CIRS elastography phantom.
Four instances of the propagation of the wave are displayed in panels A to D.
The use of ultrafast imaging allowed Macé et al. (2011) to first describe the technique of ultrasonic functional imaging to determine activity in the brain. The technique uses plane waves to sonify large areas of the brain at high frame rates (i.e., several kHz). Then for each pixel in the image, the Doppler signal from the blood motion in small arteries and capillaries is isolated. By using the Doppler power signal the authors were able to estimate the fluctuations of blood volume in the brain tissue. The authors demonstrated that stimulation of the whiskers in a rat correlated with an increase in Doppler signal (due to increased blood flow) in the corresponding sensory area of the brain. Since then, other authors have continued to develop this technique with its first use in humans in the preoperative setting by Imbault et al. in 2017. Later the same year, the first non-invasive application in humans was published by Demene et al (2017) to study the brain activity of newborns during sleep (Demene et al., 2017).
Figure 3 illustrates how functional imaging can be used to infer neural activity related to language processing inside the human brain. These images were acquired during awake brain surgery. The Doppler signal, available per pixel, was correlated with the stimulation signal (a word-repetition task, performed both verbally (left panel) and silently (right panel)) by the subject during surgery. These unique images reveal how different functional brain areas are involved in different aspects of language processing and production. In their study, the researchers acquired continuous ultrafast images using a Verasonics 256 system with 12-16 compounded plane wave angles at a pulse repetition frequency ranging from 6-8 kHz, for a period of 60 seconds per measurement. The live Doppler frame rate ranged between 3.6 and 4.8 Hz. These images were obtained by the CUBE research group in Rotterdam, The Netherlands. Further information regarding this study by Soloukey et al. can be found here.
The researchers’ ability to distinguish these different functional areas opens up new and exciting opportunities to study and understand how the human brain processes and produces language in health and disease.
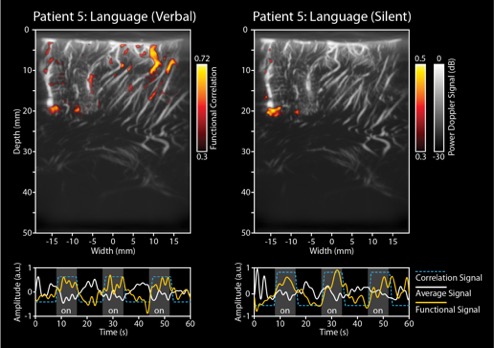
Figure 3: Functional images acquired in an intra-operative setting in order to study language processing in the human brain. The left panel depicts the functional response in the brain when the patient is verbally repeating a word, while the right panel depicts when the patient is imagining the repetition of the word, without actually pronouncing it.
Moreover, functional ultrasound imaging has been used to study brain connectivity by correlating the ultrafast Doppler signal between different centers in the brain. This methodology establishes the areas in the cortex that are working in synchronicity (coherently). In 2014 Osmanski et al, published the first work describing this technique to identify functional connectivity patterns in anesthetized rats. Figure 4 shows a modified figure from their publications allowing visualization of the functional connectivity of different areas of the rat brain for a particular coronal plane. Further information regarding this study by Osmanski et al. can be found here.
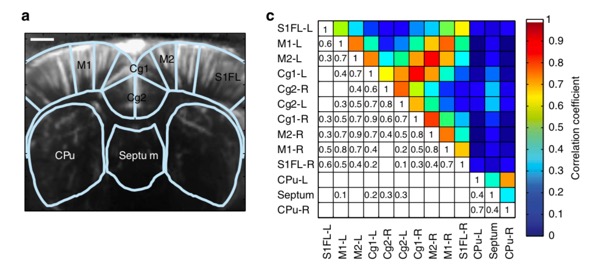
Figure 4: Functional connectivity of an anesthetized rat brain (Bregma + 0.84 mm). Panel A shows a functional image of the brain and areas of the brain that were analyzed. Panel C represents the correlation matrix and the corresponding coefficients between the different areas. Rights and Permissions license.
Lastly, in recent years ultrafast imaging has made an impact in volume imaging. Further advances in electronics and probe technology have allowed development of matrix transducers with very high numbers of elements (i.e., more than 1000 elements) as well as ultrasound systems to support a high number of channels. A publication by Provost et al. in 2014, demonstrated the application of ultrafast imaging into 3D B-mode imaging, shear-wave elastography and color Doppler (Provost et al. 2014). This study used a probe with 1024 elements produced by Vermon (Tours, France) and a system with the same number of channels to drive the probe.
Other developments using ultrafast acquisitions for volume imaging have focused on using ultrasound systems with a limited number of channels to drive matrix probes with high numbers of elements. Last year at the International Ultrasonics Symposium (IUS 2019), Verasonics introduced the concept of Sparse Random Aperture Compounding (SRAC). The method consists of using a multiplexer to transmit and receive using a random selection of the elements available in a matrix probe. Then the user can combine acquisitions with complementary element sets to reconstruct an image using all elements in the probe. This technique was used in a Vantage 256 system to drive a Vermon matrix probe with 1024 elements using a 4-to-1 multiplexer. Figure 5 shows a sequence of images of an aneurysm phantom acquired using multi-angle compounding and SRAC.
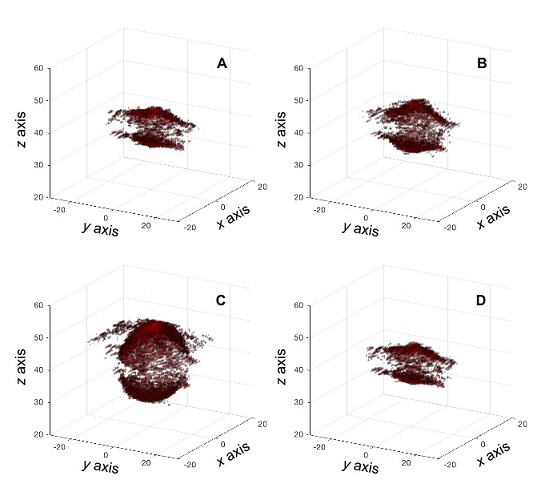
Figure 5: Ultrafast imaging of an aneurysm phantom using SRAC with a Vantage 256 system and 1024 matrix probe. Panels A-D show the deformation of the aneurysm within a pressure cycle.
Verasonics is committed to supporting ultrafast imaging for a variety of research purposes. Verasonics’ CTO, Ron Daigle, was instrumental in the early developments done by Fink et al, leading to the use of ultrafast imaging for shear wave detection (Sandrin et al., 1999, Tanter et al., 2002 and Bercoff et al., 2004).
The entire family of Vantage systems (32LE, 64, 64LE, 128, 256 and Multisystem Package [1024 channels]) supports data acquisition rates up to 100 kHz. Each channel in the Vantage system can store up to 32 MB to enable pixel-oriented reconstruction, and the Vantage software is designed to easily allow the user to perform angle compounding to improve contrast and SNR for many applications.
For more information and product technical descriptions, please visit our website or send us an email.
References
-
-
- Bruneel, C.; Torguet, R.; Rouvaen, K.M.; Bridoux, E.; Nongaillard, B. Ultrafast echotomographic system using optical processing of ultrasonic signals. Appl. Phys. Lett. 1977, vol. 30, pp. 371–373.
- Daigle, R., Verasonics Inc, 2012. Ultrasound imaging system with pixel oriented processing. US Patent number US8287456B2.
- J. Cheng and J.Y. Lu, Extended high frame rate imaging method with limited diffraction beams. IEEE Trans. Ultrason. Ferroelectr. Freq. Control, vol. 53, pp. 880–899, May 2006.
- Montaldo, G.; Tanter, M.; Bercoff, J.; Benech, N.; Fink, M. Coherent plane-wave compounding for very high frame rate ultrasonography and transient elastography. IEEE Trans. Ultrason. Ferroelectr. Freq. Control. 2009, 56, 489–506.
- L. Sandrin, S. Catheline, M. Tanter, X. Hennequin, and M. Fink, Time-resolved pulsed elastography with ultrafast ultrasonic imaging, Ultrason. Imaging, 1999, vol. 21, no. 4, pp. 259–272.
- J. Bercoff, M. Tanter, and M. Fink, Supersonic shear imaging: A new technique for soft tissue elasticity mapping, IEEE Trans. Ultrason. Ferroelectr. Freq. Control, 2004, vol. 51, no. 4, pp. 396–409.
- E. Mace, G. Montaldo, I. Cohen, M. Baulac, M. Fink, and M. Tanter, Functional ultrasound imaging of the brain, Nat. Methods, 2011, vol. 8, no. 8, pp. 662–664.
- Marion Imbault, Dorian Chauvet, Jean-Luc Gennisson, Laurent Capelle, Mickael Tanter. Intraoperative Functional Ultrasound Imaging of Human Brain Activity. Scientific Reports, Nature Publishing Group, 2017, 7 (1), pp.7304.
- Demene, C., Baranger, J., Bernal, M., Delanoe, C., Auvin, S., Biran, V., Alison, M., Mairesse, J., Harribaud, E., Pernot, M., Tanter, M., Baud, O., Functional ultrasound imaging of brain activity in human newborns. Sci. Transl. Med. 2017 9, eaah6756.
- Soloukey S, Vincent AJPE, Satoer DD, Mastik F, Smits M, Dirven CMF, Strydis C, Bosch JG, van der Steen AFW, De Zeeuw CI, Koekkoek SKE and Kruizinga P. Functional Ultrasound (fUS) During Awake Brain Surgery: The Clinical Potential of Intra-Operative Functional and Vascular Brain Mapping. Front. Neurosci. 13:1384, 2020. doi: 10.3389/fnins.2019.01384.
- Osmanski, B. F., Pezet, S., Ricobaraza, A., Lenkei, Z., and Tanter, M. Functional ultrasound imaging of intrinsic connectivity in the living rat brain with high spatiotemporal resolution. Nat. Commun. 5:5023, 2014. doi: 10.1038/ncomms6023.
- Provost, J., Papadacci, C., Arango, J.E., Imbault, M., Fink, M., Gennisson, J.L., Tanter, M., Pernot, M., 3D ultrafast ultrasound imaging in vivo. Phys. Med. Biol. 2014, 59, L1–L13. https://doi.org/10.1088/0031-9155/59/19/L1.
- M. Tanter, J. Bercoff, L. Sandrin, and M. Fink, Ultrafast compound imaging for 2-D motion vector estimation: Application to transient elastography, IEEE Trans. Ultrason. Ferroelectr. Freq. Control, 2002, vol. 49, no. 10, pp. 1363–1374.
-
During the COVID-19 crisis, we are here for you. Verasonics offers ultrasound simulator software that can assist in the continuation of research in a remote setting. Vantage customers have access to five simulator licenses with their system purchase that enable programming and verification of custom acquisition sequences without Vantage system hardware present. This allows for sharing of a single hardware system among multiple users and allows research and development teams to work virtually. To expand your lab’s efficiencies while working remotely, Verasonics is offering increased access to the ultrasound simulator software to all customers. Verasonics is pleased to provide an additional five ultrasound simulator software licenses per account on a temporary basis (expires in 6 months). It is our sincere hope that we can support your goals and research during this unsettling time. For more information, please send us an email.
Visit Us and Learn What’s New at these Upcoming Conferences:
Currently there are no upcoming conferences, however, please join us for our next Vantage customer training via live webinar, 1-4 February, 2021, 9 – 11 am PT.
Please contact [email protected] to register or click here for more information.